general comments
suspended solids and colloïds
definitions
Please refer to the section the physical states of impurities in water, groundwater and sections seawater and brackish water and removing hardness (calcium and magnesium) for explanations and discussions on the three categories of impurities whose elimination is targeted by water treatment:
- suspended solids (sand, silt, plankton, organic debris…);
- colloidal matter (fine clay, protozoa cysts, bacteria, macromolecules...);
- dissolved matter (OM, salts, gases…);
the first two are responsible for turbidity, the latter two for colour and the last one for salinity and various other water properties.
role played by coagulation-flocculation
Coagulation and flocculation processes are used to facilitate the elimination of suspended solids and colloids by gathering these together to form floc. Sedimentation, flotation and/or filtration systems are then used to separate the floc (see the section sedimentation, filtration, as well as flocculators – settling tanks – flotation units and filters).
The above constitute the fundamental treatments applied with the goal of rectifying all or part of the defects found in water and caused by inert (silt, clay, colloids) or living (plankton micro-algae; micro-invertebrates and especially parasitical protozoa cysts) particulate fractions amoeba, Giardia, Cryptosporidium…; bacteria); these treatments are also responsible for removing the fraction of organic matter "capable of flocculation" (macromolecules, and especially most humic acids responsible for colouring water), some heavy metals, more generally, the fraction of micro-pollutants associated with these suspended solids and colloidal macromolecules (including viruses, that are almost always carried by the suspended solids and colloids found in water).
colloidal suspensions – the need for coagulation
colloidal suspension stability
Table 1 lists some matter or organisms with their dimensions and the timescale required by these particles to fall vertically through one metre of water at 20°C, solely under the influence of their weight.

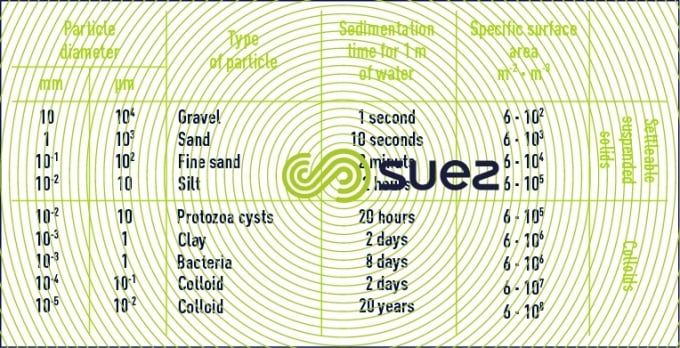

(see section different types of sedimentation)
Table 1 demonstrates that colloids are particles:
- that cannot be settled naturally;
- that have a very large specific surface area that governs their suspension stability in water.
In fact, in order to obtain faster sedimentation rates, a very large number of colloids must be gathered together to form aggregates measuring at least 10 to 100 mm; however, these colloids apply an electrostatic type repulsion force against each other, preventing them from agglomerating : consequently, their suspension can remain quite stable.
twin layer theory
Colloids present in raw water usually have very high negative charges (due to flaws in the crystalline network, and ionisation affecting peripheral chemical groups). In order to neutralise this negative surface charge, positive ions (called “counter ions”), present in or added to raw water, are attracted and gather to form a layer around the colloid. A range of theories have been put forward (figure 1):
- the Helmholtz theory: the colloid surface is completely encased in a layer of positive ions thus ensuring that the assembly remains neutral (attached layer);
- the Gouy-Chapman theory: a layer of positive ions is unevenly distributed round the colloid; neutrality is achieved at a greater distance (diffuse layer);
- the Stern theory that combines the two foregoing theories and entertains the formation of a twin layer: the first is formed of ions from the liquid and adheres to the colloid; the second layer diffuses through the liquid that directly surrounds the colloid. As illustrated in figure 1 (curve 3), the potential is subject to a major initial drop in the attached layer and then decreases slowly as the distance increases, until it cancels out at point A (isoelectric point).

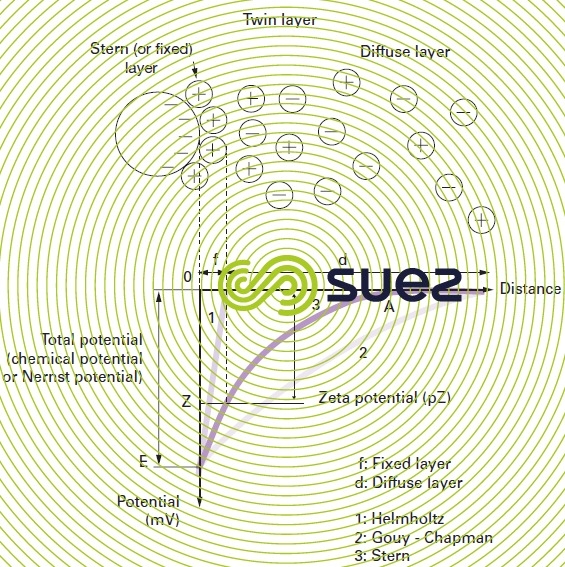

the zeta potential
A colloid has two potentials (figure 1):
- E: Thermodynamic potential also known as the Nernst potential, which is present on the actual surface of the colloid but which cannot be measured using simple methods;
- Z: Potential on the surface of the attached layer also known as the electrokinetic potential or Zeta potential (pZ). As already stated, this potential remains negative. The charge in the ions on the attached layer does not balance out the negative charges on the colloid surface. This potential governs the reciprocal interaction between colloids and can be measured through electrophoresis; in effect, when a colloid is subjected to an electrical field, it reaches a velocity such that a balance is established between the electrical attraction force to the anode and the friction force created by the medium’s viscosity. The equation linking this velocity (electrophoretic mobility) and the Zeta potential is written as follows:

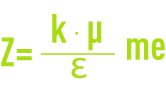
me: electrophoretic mobility (µm·s–1·V–1),
Ɛ: medium’s dielectric constant,
μ: dynamic viscosity (Pa.s),
k: based on particle diameter and on twin layer thickness.
It should be noted that, by definition, particles that have the same Zeta electrokinetic potential will have the same electrophoretic mobility, regardless of their diameter.
The Zetameter is the apparatus used to measure Zeta potential (see treatability tests section).
colloidal suspension destabilisation mechanism: coagulation
When two colloidal particles approach each other, they are subjected to two major types of force acting in opposing directions (figure 2):

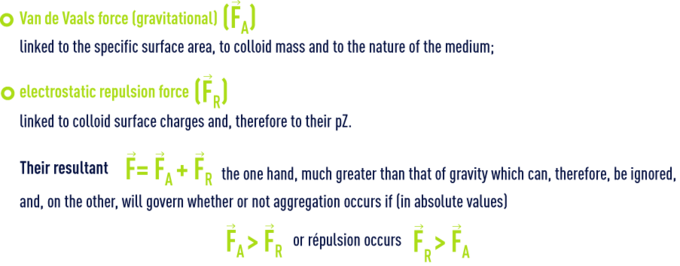
The second case (repulsion) is the one found in natural water, hence the stability of colloidal suspensions. On the right hand side of figure 2, we can see that the change in the resultant force creates an “energy barrier” in the vicinity of the particles.

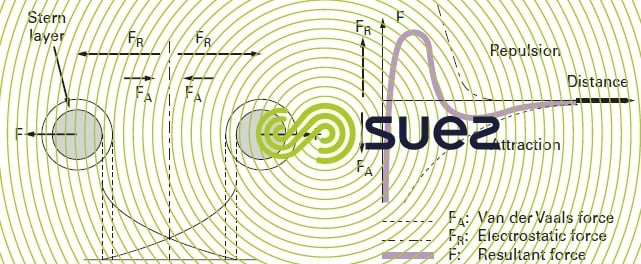

Therefore, in order to destabilise the suspension (coagulation), we need to decrease electrostatic repulsion forces and this involves neutralising colloid surface charges: this is what happens when we add a so called “coagulant” into the water (figure 3 and explanations in general comments).
In the twin layer theory, optimum coagulation can be defined as adding a reagent that enables the Zeta potential to be cancelled out.

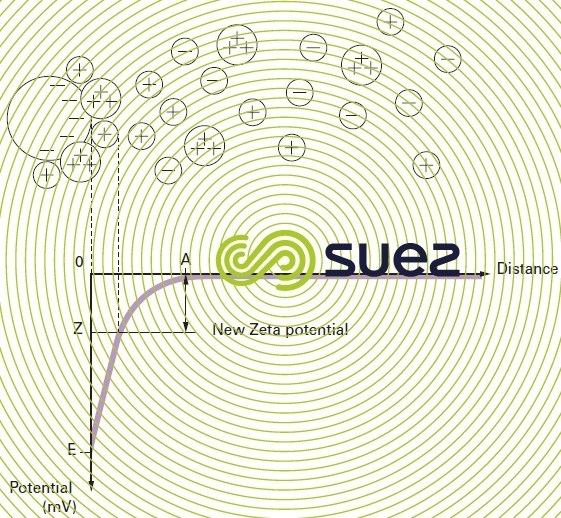

aggregations stages
factors that influence coagulation
Therefore, coagulation is the destabilisation of colloidal particles though addition of a chemical reagent, the coagulant, which provides the medium with multivalent cations that are either free or bonded to an organic macromolecule (cationic polyelectrolyte). These cations are adsorbed and attached in the first Stern layer; the pZ then rises (figure 3) until it reaches zero or a negligible figure as neutralisation of all the particle’s electronegative charges is achieved (figure 7, see section treatability tests).
It should be noted that, in order to be effective, the coagulant must be dispersed immediately through the water in order to achieve an even distribution and that this must occur before any hydroxide precipitates. To achieve this, we need to dissipate a high level of energy over a short time or, in other terms, use a very steep velocity gradient.
Under turbulent conditions, the velocity gradient is defined by the following formula:

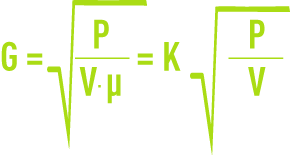
G: mean velocity gradient (s-1),
P: power actually dissipated (W),
V: space occupied by the fluid (m3),
µ: dynamic viscosity (Pa.s),
G is particularly dependant on temperature via the constant K (table 2).

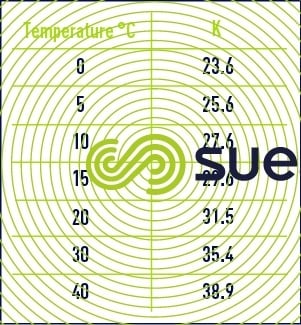

flocculation
This is when particles agglomerate (after having “been neutralised”) into micro-floc through bridging or assisted by hydroxides produced as the mineral coagulant hydrolyses or by means of the macromolecules in the cationic polyelectrolyte. Microfloc then comes together to form increasingly voluminous flakes that can settle, floc. This flocculation can be enhanced by adding another reagent: the flocculation additive, more simply known as the flocculant.
In fact, the successive aggregation steps that lead to the formation of floc depend on two transport phenomena that govern flocculation rate:
- perikinetic flocculation linked to the Brownian diffusion (thermal agitation) where all particles have the same kinetic energy and, therefore, the smallest have the highest velocities, ensuring a greater probability of the flocs coming together. Flocculation rate or the variation in the number of particles that have agglomerated over a period of time is provided by:

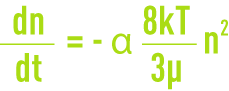
n: number of particles per unit of volume,
α: effective collision fraction,
k: Boltzmann constant,
T: absolute temperature.
This law only applies to small particles measuring less than 10 mm. This law describes how microfloc is formed; we need to note the effect of particle “density” (n) and of temperature;
- orthokinetic flocculation is related to the mechanical energy dissipated in the flocculation zone. Please refer to table 3 for details on the effectiveness of this flocculation which produces a voluminous floc that can be separated.

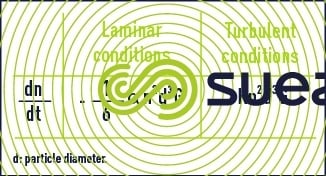

The velocity gradient is also a very important flocculation rate parameter.
In flocculation, the velocity gradient acts on the floc aggregation probability, but cannot excessively increase this probability. Indeed, when G reaches excessively high figures, the floc that has formed will undergo mechanical shear, causing its destruction. The normally acceptable values for G are:
- in coagulation: 400, or even 1,000 s–1;
- in flocculation: approximately 100 s–1 and less as soon as floc size exceeds one millimetre.
coagulation and flocculation times
The coagulation time is typically measured in seconds whereas flocculation time is typically measured in minutes (typical example: 3 seconds - 20 minutes).
The application of the flocculation reaction can be characterised by the G·ξ (ξ = contact time) a dimension less parameter. A flocculation test can be used to determine the value of ξ (see section measuring global parameters).
summing up the phenomena
Table 4 summarises the various successive or simultaneous phases leading to the formation of floc from a colloidal suspension.

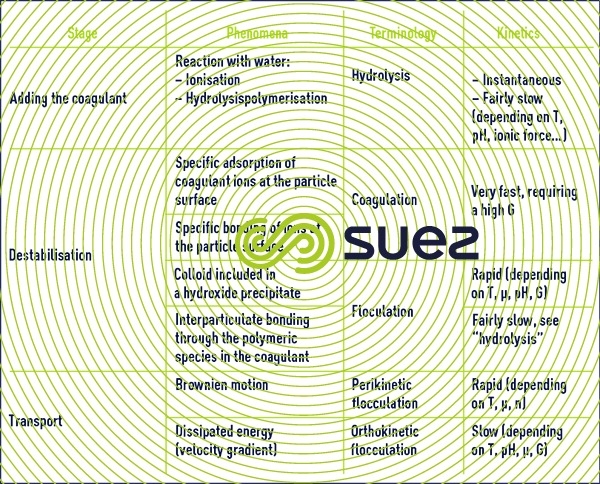

coagulants
trivalent cations
Coagulation becomes even more efficient as the cation valency rises (Schulze-Hardy theory) :

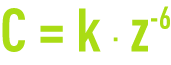
where C = reagent demand,
z = valency of the counter ion used;
thus, a trivalent ion will be approximately ten times more effective than a divalent ion. Also, in practice, trivalent aluminium or iron salts have been and continue to be widely used in all water coagulation treatments.
effect of the pH
As a result of their hydrolysis, mineral coagulants alter the physical-chemical properties of the water to be treated (pH, M-alk., conductivity):

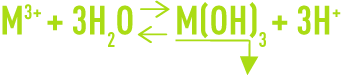
Furthermore, the optimum pH is a compromise between:
- the pH required for coagulation (linked to the nature of the colloids and to their isoelectrical point);
- the pH required for flocculation (linked to the growth of the aluminium or iron hydroxide floc – table 5). In general, it refers to the minimum solubility of the hydroxide concerned (also required in order to ensure that the minimum amount of metal is dissolved in water, according to applicable potability standards).

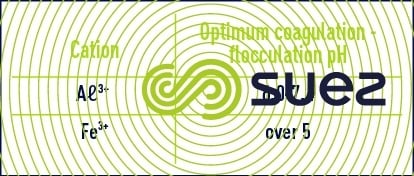

This pH and minimum solubility are strongly affected by the ionic force and by the presence of organic compounds such as humic acids.
If necessary, an acid or a base will have to be used to adjust the coagulation pH.
treatment rate
A flocculation test will establish the appropriate treatment rate. It can be adjusted through a study of the Zeta potential (see section the benefits of membrane bioreactors).
"Ideal" coagulation occurs at pZ = 0, ensuring optimum elimination of all particles (clay turbidity, micro algae…); in drinking water treatment, the thorough elimination of dissolved OM may require a higher level of treatment. An amount of coagulant that is progressively higher than that which will cancel out the pZ will initially cause residual turbidity to appear (figure 4, using arbitrary units = UA) by reversing charges (trivalent cations cause pZ to become positive) before removing this turbidity by capturing the colloids in the excess floc ("sweep coagulation"; this coagulation mode is sometimes applied while at the same time using H2SO4 to lower the pH ("enhanced coagulation", applied in particular to ensure thorough elimination of the organic precursors of oxidation by-products).

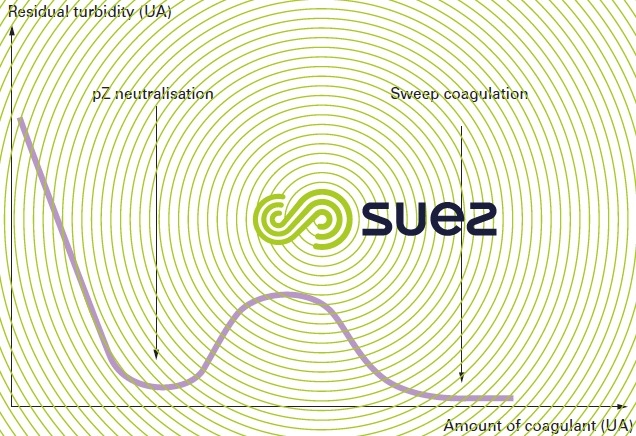

On the other hand of the scale, the term "micro flocculation" is sometimes used in the sense of a short flocculation period used to form small size floc measuring 1 to 2 mm in diameter, but sufficiently voluminous to allow the floc to be separated in a flotation unit or through filters. In direct filtration mode, a reduced amount of coagulant may also be used.
sludge production
The formation of metal hydroxide leads to the production of large amounts of sludge. In its untreated form, this sludge is rarely suitable for discharge into the natural environment and, therefore, must be treated (see chapter liquid sludge treatment and section sludge processing).
organic coagulants
When sometimes used instead of or in addition to mineral coagulants, these cationic polymers will neutralise negative colloids directly through their positive charges. Their adsorption to the surface of these colloids will allow a bridging effect and, therefore flocculation, to occur at the same time. One of the main benefits will be the significantly reduced volume of sludge produced (no hydroxides).
flocculation additives (or flocculants)
In order to enhance flocculation, mineral (activated silica) or natural (starch, alginate) polymers were first used. However, the appearance of synthetic polymers (very long macromolecules that tend to be adsorbed over microfloc and, therefore, to connect them together) has led to major developments in flocculation performance, creating larger floc that has greater shear resistance.
As in the case of the coagulant, the optimum treatment level required will be determined by flocculation tests (designated jar-test, see section specific analysis) supplemented, if necessary, by sludge settleability tests.
The interval separating the injection of coagulant and the injection of flocculant is crucial: In effect, a flocculant is only effective when the micro-flocculation phase has been completed. This interval is based on a number of factors (water composition, temperature…) and must be established through experimentation in each case.
The use of synthetic flocculants produces far lower volumes of sludge.
effect of pre-oxidation
Prechlorination (e.g. in seawater) and in particular, pre-ozonation have been recognised as assisting the coagulation-flocculation mechanism. At this point, we can refer to the destruction or, at least, the desorption of the organic film that surrounds some colloidal particles and thus prevents the destabilising cations from attaching. Moreover, low amounts of ozone (< 1 g · m–3) will reduce the number of particles and increase the gramme-molecular weight of some organic compounds (reticulation), that will also have favorable action on coagulation. This is typically the case of water that is rich in organic matter and algae or in organic matter that sequesters iron or manganese; in the latter case, ozone will destroy organic complexes and oxidise the metal ions that are thus released.
Bookmark tool
Click on the bookmark tool, highlight the last read paragraph to continue your reading later
